- DOI 10.31509/2658-607x-202251-100
INFLUENCE OF VEGETATION ON SOIL CARBON STOCKS IN FORESTS (REVIEW)
С текстом оригинальной статьи Вы можете ознакомиться по ссылке
Original Russian Text © 2021 А. I. Kuznetsovа published in Forest Science Issues Vol. 4, No. 4, Article 95
A. I. Kuznetsovа
Center for Forest Ecology and Productivity of the Russian Academy of Sciences,
Profsoyuznaya st. 84/32 bldg. 14, 117997, Moscow, Russia
E-mail: nasta472288813@yandex.ru
Received 15 November 2021
Revised 17 December 2021
Accepted 18 December 2021
Existing estimates of carbon stocks in taiga and coniferous-broadleaf forests show that almost half of the total organic carbon in these ecosystems is accumulated in forest soils. Vegetation as the main source of organic matter in the soil interacts with soil biota, which processes plant litter, and, with abiotic environmental factors, determines the processes of formation and accumulation of soil organic matter. Changes in the composition of vegetation are the driver of the dynamics of soil carbon stocks; however, insufficient attention has been paid to the analysis of this issue. This review analyzes the main ways of transferring carbon from the vegetation pool to the soil pool and the influence of three main predictors of vegetation that affect the carbon stock in soils: the amount and quality of litter of individual plant species (species identity) and the structural diversity of the plant community; it identifies gaps in knowledge and proposes some ways of developing this field of research.
Keywords: vegetation, soil organic horizon, soil, carbon stock, litter quality, litter amount, interbiogeocenotic heterogeneity, intrabiogeocenotic heterogeneity
Climate change is one of the current global challenges. Forests play a huge role in climate regulation due to their ability to absorb greenhouse gases and store carbon in both biomass and soils. The proportion of soil carbon in the total carbon stocks of forests is 40% or more (Framstad et al., 2013). Over the past 10 years alone, several meta-analyses have been conducted on a regional and global scale to identify the main factors of carbon accumulation in the soil.
When assessing soil carbon stocks on a regional scale, the leading role of climate is highlighted (Wiesmeier et al., 2019). Local abiotic factors that have an impact on the level of carbon accumulation in soils include granulometric and chemical composition of soil-forming rocks, as well as topographic features of an area. Special focus is on the study of anthropogenic factors, that is, the influence of forestry regimes in the past and present, the influence of forest fires, and the history of forest development (Framstad et al., 2013; Mayer et al., 2020).
Vegetation, fauna and microbiota are the main biotic factors of soil carbon accumulation. The influence of biota on soil carbon stocks can be considered at different spatial levels, taking into account natural borders (forest catchments of different orders, forest type, etc.) and administrative (national, regional and local) boundaries.
At high spatial (e. g., regional) levels, the relation between soil carbon stocks and climatic conditions was revealed: there are positive correlations with average annual temperature, average annual precipitation and, accordingly, net primary productivity (Amundson, 2001). Comparing different climatic zones showed a decrease in carbon stocks of the litter pool and an increase in carbon stocks in the mineral layers of the soil from north to south (Wiesmeier et al., 2019).
At all spatial levels, the correlation with vegetation was found. Special emphasis was put on the influence vegetation has on organic matter decomposition in forest ecosystems (Prescott, 2010, Krishna, Mohan, 2017; Berg, McClaugherty, 2020, Ivanova, 2021). Some studies analyze stabilization processes of soil organic matter of microbial and plant origin (Angst et al., 2021) and dissolved organic carbon in forest soils (Karavanova, 2013).
Vegetation composition determines the quantity and quality of incoming plant litterfall, its transformation and transition to soil pools with the active participation of decomposers, as well as migration processes of carbon compounds within the soil profile (Gleixner, 2013; Krishna, Mohan, 2017). For boreal and subboreal forests, differences in carbon stocks in forests of different types were shown (Jandl et al., 2007; Oostra et al., 2006; Schulp et al., 2008; Akkumuljacija…, 2018; Kuznetsova et al., 2019, 2020), including the contribution of not only the tree layer, but also the ground cover (Lukina et al., 2020; Kuznetsova et al., 2021). The question of the combined effect of different plant species on carbon stocks remains open.
A better understanding and assessment of the combined influence of various factors on carbon accumulation in forest soils is crucial for tackling tasks like development of measures for climate change mitigation and forecasting possible changes in ecosystem functions and services. Such estimates are few, although they are of vital scientific and applied importance, especially more recently, due to the necessity to achieve carbon neutrality.
The purpose of this review is to summarize existing knowledge about the influence of vegetation-related factors on the dynamics of soil carbon pools.
- Major mechanisms of accumulation of soil organic matter
1.1. Major mechanisms of carbon transfer from the vegetation pool to the soil pool
Soil carbon stocks result from organic matter entering the soil and carbon compounds being lost due to decomposition, washing out and leaching (Fig. 1). The source of organic matter is the plant material of both aboveground and underground organs. Other sources of carbon compounds are root secretions, including exudates of plant roots and associated symbionts (for example, mycorrhizal fungal mycelium), as well as fecal material and bodies of soil biota. Once in or on the soil, organic matter is gradually transformed by soil fauna and microorganisms (fungi, bacteria and archaea). Extracellular enzymes secreted by the microorganisms break down the organic matter into simpler compounds that can be assimilated. Part of the plant organic matter is mineralized, and the other part is accumulated in the biomass of consumers and decomposers or in their metabolites, which can partly be excreted from the cells and stabilized. The rest of some stable plant biomolecules, including lipids, lignins and sugars, can also be stabilized by minerals and aggregates.
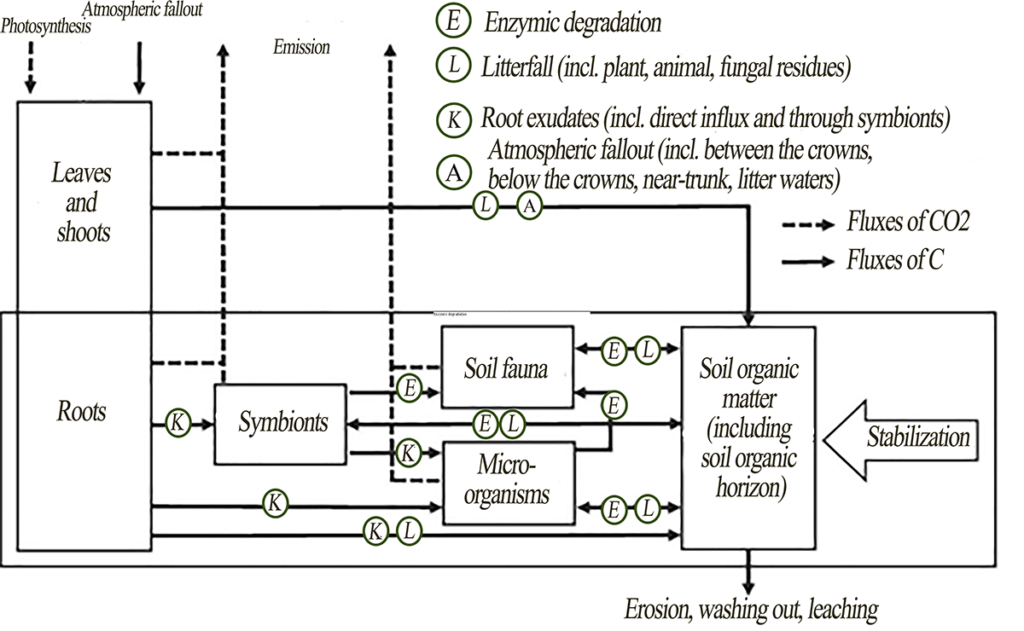
Figure 1. Carbon fluxes in a forest ecosystem acc. to Mayer et al. (2020), modified
Since vegetation is the main source of organic matter, it is important to discuss the principal mechanisms of carbon transition from the vegetation pool to the soil pool. Three main mechanisms were identified.
- Processing of aboveground and underground litterfall by soil fauna and microbiota. Soil fauna mechanically destroys and mixes the litterfall with the soil organic horizon and the mineral part of the soil; therefore, it can contribute to the spatial redistribution and primary destruction of plant material (Brussaard, 1997; Frouz et al., 2013). As decomposers, especially microbiota, further process the soil organic matter (SOM), the soil organic horizon undergoes chemical and structural changes (Frouz, 2018). For example, earthworm activity can both enhance the transformation of soil organic horizon into more stable organomineral aggregates and contribute to the accelerated SOM degradation (Wolters, 2000; Fox et al., 2006), which can be taken into account when identifying functional types of macrofauna (Geraskina, 2020).
The transformation of litterfall by soil biota differs significantly under different climatic conditions, since temperature and water regimes are the limiting factors for many decomposers. In boreal forests of the Northern Hemisphere with low temperatures and humid water regime, fungal decomposition usually prevails, which contributes to litter accumulation and formation of raw forms of humus such as mor and moder. In southern regions, biological cycle increases in intensity, and, together with fungal decomposition, the proportion of bacterial decomposition and contribution of soil animals increases, thus contributing to a more intensive litter decomposition and humification of the soil organic horizon and upper mineral horizons.
- Vertical stratification of tree roots (Brassard et al., 2011), root litter (Brassard et al., 2013) and root turnover (Brassard et al., 2011; Lei et al., 2012), as well as root exudates (Bardgett, 2005), contribute to the successful association of organic matter with soil aggregates or clay minerals. Some researchers suggest that most of the SOM originates from roots, and the deeper they are, the more significant this source becomes (Rasse et al., 2005), since different types of root systems make vertical stratification of tree roots and redistribution of the rhizosphere into deep mineral horizons possible. Root litter usually decomposes slower than foliar litter of the same species (Lauenroth, Gill, 2003; Cusack et al., 2009); therefore, more stable carbon compounds of roots stay in the soil longer than carbon compounds of aboveground shoots (Rasse et al., 2005). The key mechanisms that lead to root carbon stabilization in the soil include: (1) chemical resistance of roots, partly due to the presence of a persistent compound suberin; (2) physical protection in aggregates; and (3) physico-chemical protection of lignin and reactive carbon compounds in the soil (root exudates) associated with clay minerals. However, the opposite effect is also possible: labile C isolated from the roots may stimulate the decomposition of the already stable SOM (Kuzyakov, Domanski, 2000; Dijkstra, Cheng, 2007).
When comparing different biomes, global patterns in the rate of root system renewal between plant groups and depending on climatic gradients were established. Since the rate of root renewal increases exponentially along with an increase in the average annual temperature (Gill, Jackson, 2000), a more significant contribution of root litter to carbon stabilization can be expected in southern regions.
- Leaching of dissolved organic carbon (DOC) from living plants and forest soil organic horizon is yet another way for carbon compounds to go into deeper soil horizons (Fröberg et al., 2011). DOC leaching fluxes are usually higher directly under the forest soil organic horizon and are often associated with microbial activity and biomass (Smolander, Kitunen, 2002). Also, high DOC fluxes into the mineral part of the soil are found in forests with high carbon content in the soil organic horizon, for example, those formed by common spruce. Using the example of spruce forests in the European part of Russia, it was shown that, at a soil solution carbon concentration of 35 mg/L and above, water-soluble organics (WSOs) begin to be sorbed in the BF horizon (Karavanova et al., 2020). In dissolved organic substances moving down the soil profile, lignin derivatives (phenols) are mainly preserved on the surface of oxides and hydroxides in shallow soil layers (Kaiser et al., 2004; Kaiser, Zech, 2000). DOC moving from upper to deeper soil horizons can result in stabilization and, consequently, a significant increase in soil carbon deposition (Kalbitz, Kaiser, 2008). This can be pronounced against the background of excessive moisture, when an increase in the flux of plant-derived DOC and its consolidation are possible (Mikutta et al., 2019). However, an inverse effect is also possible: estimates of DOC removal from temperate pine forests in Belgium (about 10 g/m2 per year) have shown that DOC can account for 11% of ecosystem net productivity (Gielen et. al., 2011).
Carbon fluxes in forests can differ significantly in the north and in the south, since it is known that climatic conditions can have a significant impact on both the amount of DOC and intensity of its removal (Gmach et al., 2020). On the one hand, the biological cycle intensity is higher in southern regions than in northern ones, which contributes to active decomposition of the soil organic horizon and DOC being released. On the other hand, during prolonged dry seasons, decomposition rate of the soil organic horizon may decrease, and secondary metabolites may accumulate (Kalbitz et al., 2000).
Therefore, three main ways of transferring carbon from the vegetation pool to the soil pool were identified. At the same time, there are no estimates of the contribution of each of these mechanisms or their combined impact. It was found that, in the northern and southern regions (of the Northern Hemisphere), the intensity of these processes manifests in different ways. It is expected that in northern ecosystems the contribution of DOC to organic matter accumulation will be high, since these areas have a high level of precipitation and highly developed soil organic horizon. The predominance of fungal decomposition will also contribute to the accumulation of the soil organic horizon. At the same time, the influence of root litter can be very significant, especially if we take into account the influence of not only woody plants, but also that of shrubs widespread in taiga forests and other ground cover vegetation. In the southern coniferous-broadleaf forests, DOC fluxes are much smaller than in taiga, because there is less precipitation. However, an increase in biomass and activity of soil fauna increases its contribution to litter processing and subsequent stabilization of SOM in the mineral part of the profile. An increase in overall productivity of roots, along with influence of saprophages, is probably the key mechanism for a more intensive formation of stable carbon in the mineral horizons of soils found in coniferous-broadleaf forests. However, all these assumptions are hypothetical and require further research.
1.2 Main mechanisms of carbon fixation in the SOM
Carbon sequestration in soils is hardly a new aspect in the studies of biogeochemical carbon cycle within the framework of the global climate change and terrestrial ecosystems: as early as in the 1990s some studies provided evidence of the importance of soils as a carbon sink under conditions of elevated CO2 concentration in the atmosphere.
Carbon sequestration capacity of the soil (Carbon Protection Capacity, CPC) reflects its ability to stabilize and retain the carbon that came with organic matter as part of soil organic matter (SOM) (Semenov et al., 2009; Kogut, Semenov, 2020).
It is now agreed that there are two main mechanisms of organic matter stabilization in soils: due to the formation of organomineral complexes (Semenov, Kogut, 2015) and due to the formation of soil aggregates (Six et al., 2002, 2004; von Lützow et al., 2006; Gunina et al., 2015).
Organomineral complexes are formed as a result of physicochemical interaction between molecules of soil organic matter (OM) and mineral components, increasing the protection of this SOM (soil organic matter) from decomposition (von Lützow et al., 2006). For example, these include the formation of high-molecular humic substances; binding of hydrophilic components of fresh organic matter by hydrophobic centers of humic substances, and binding of amino acids by polyphenols; formation of organomineral complexes of aluminum and iron, calcium; and formation of organomineral complexes in mineral matrices of clay particles. These processes mainly involve fine clay and mineral particles, with the high contribution of surfaces of chemically reactive oxides and phyllosilicates (Blume et al., 2015).
Soil aggregates are homogeneous mixtures of minerals, organic compounds and organomineral complexes. For example, microaggregates can prevent microorganisms from getting access to SOM inside the aggregates, increasing its stability (Six et al., 2004). Accumulation of dissolved organic carbon in pores that are smaller than bacteria is also possible (Totsche et al., 2018).
There is also the third, currently widely discussed mechanism for OM stabilization, i. e. biochemical protection due to certain chemical properties of biomolecules. It was established that in forest ecosystems the major part (≥50%) of stabilized carbon is plant biomolecules, including lipids, lignin and sugars, which can make up a significant part of organic matter protected by minerals and aggregates (Angst et al., 2021).
Biochemical resistance is believed to potentially be most relevant at the initial stages of decomposition and possibly play only a minor role in the long-term protection of SOM in mineral soil (Marschner et al., 2008). Some biomolecules can, however, be “selectively preserved” depending on the thermodynamic conditions of the environment and their ability to be stabilized due to physicochemical interactions in the soil matrix: for example, this is true of lignin (Feng et al., 2005), some sugars (Amelung et al., 1999; Kiem, Kögel-Knabner, 2003) and lipids (Angst et al., 2017b; Ludwig et al., 2015).
The intake and subsequent stabilization of aromatic and aliphatic acids, such as lignin monomers and its oxidation products, depend on the dominant vegetation. Guaiacyl (vanillin) phenols dominate in coniferous forests (Kovalev, Kovaleva 2016); they persist longer during the initial decomposition processes and have a higher probability of binding to mineral surfaces (Clemente, Simpson, 2013). Broadleaf and small-leaved forests contain equal proportions of vanillins and syringils (Kovalev, Kovaleva 2016). The main source of aromatic phenolic compounds in soils is lignin from aboveground and underground biomass of higher plants. Underground parts of plants play the main role (Kovalev, Kovaleva, 2016). The number of studies on the extraction of lignin from intact aggregate structures is small. Few studies indicate higher lignin content and a low degree of lignin oxidation in macroaggregates as compared to microaggregates (Xiao et al., 2007; Thevenot et al., 2010). The participation of lignin phenols in aggregation and concretion formation was established depending on the redox situation of soils (Kovalev, Kovaleva, 2016).
Neutral sugars of plant origin (mainly derived from hemicelluloses) are preferred microbial substrates compared to other forms of SOM (Gunina, Kuzyakov, 2015), such as lignin, and may contribute to the accumulation of microbial necromass. Interestingly, the concentration of mostly neutral sugars of plant origin (for example, xylose and arabinose) in organomineral complexes (52–128 mg/g C) may be several times higher than the concentration of lignin (Kiem, Kögel-Knabner, 2003; Córdova et al., 2018). Neutral sugars of plant origin can make a significant contribution (up to 130 mg/g C) (Córdova et al., 2018) to the stabilization of SOM.
Some lipids of plant origin are considered relatively resistant to degradation, including long-chain n-alkanoic acids, certain monomers of cutin and suberin with groups of hydroxylic and/or carboxylic acids (Quenea et al., 2004; Jandl et al., 2005; Angst et al., 2017a; Anohina, 2020). Stabilization of plant-derived lipids through organomineral interactions may depend on the monomeric composition of lipid biopolymers and chemical properties of these monomers, as well as the composition of microbial population, sorption properties and mineral composition of soils (Bull, 2000). The contribution of plant-derived lipids to stable C ranges from ~2% to ~10% (i. e. ~ 20–100 mg of lipids per g of C (Angst et al., 2021). Studies on the extraction of these compounds from aggregates are few and suggest the presence of alkanes in the soil as part of capsules of the independent phase of lipids (Anohina, 2020). Differences in the quantitative and qualitative composition of the lipid organic profile were revealed for different types of forests.
Thus, vegetation as the main source of organic matter entering the soil determines the possibility and speed of formation and stabilization of soil organic matter. The significant direct contribution of vegetation to the accumulation of SOM is emphasized. While the mechanism of carbon stabilization due to the formation of organomineral complexes has been sufficiently studied and has quantitative estimates, the mechanisms associated with the study of the physical protection of organic matter in soil aggregates and the biochemical stability of plant compounds require attention.
- Mechanisms related to the influence of vegetation on soil carbon stocks at the ecosystem level
Taking into account the features of the biogeochemical carbon cycle in forest ecosystems (Fig. 1) and the main mechanisms for the intake and stabilization of organic matter in soils, three main mechanisms of vegetation influence on the dynamics of soil carbon pools at the ecosystem level can be distinguished: (1) litterfall quantity and (2) quality of both individual species (species identity) and their combined influence, i. e. (3) biodiversity of the community.
The amount of litterfall is an important predictor of carbon accumulation (Grandy, Neff, 2008; Gentile et al., 2011; Carrington et al., 2012; Dungait et al., 2012), since vegetation is the main supplier of organic matter to the soil. The amount of litterfall produced is proportional to the net primary productivity of forests in natural forests, since the litterfall is part of the NPP (Chen et al., 2017).
Litter quality also depends on the composition of vegetation and the content of nutrients (nitrogen, phosphorus, potassium, etc.) and secondary metabolites (polyphenols, lignins, cellulose, hemicellulose, etc.) (Berg et al., 1993; Cadisch, Giller 1997; Perez-Harguindeguy et al., 2000, Berg, McClaugherty, 2020). The concept of correlation between accumulation of soil carbon and the quality of litter has been proposed, where high-quality litter does not always contribute more effectively to an increase in stable soil organic matter as compared to low-quality litter (Castellano et al., 2015).
One of the aspects of biodiversity is structural biodiversity characterizing the spatial organization of vegetation (vertical and horizontal structure), which determines the spatial variations of carbon intake, firstly, by regulating the penetration and falling of organic residues into the organic layer, and secondly, by influencing the hydrothermal regime of the organic layer, that is, the dynamics of temperature and water content, which in turn can affect decomposition to a greater extent than differences in macroclimatic conditions on a continental scale (Joly et al., 2017). Other aspects of biodiversity (typological, species, functional, age, ontogenetic structure, etc.) also determine the dynamics of carbon intake and its quantitative and qualitative characteristics.
Let’s look into the influence of these mechanisms at different spatial levels.
2.1. Regional level
Estimates of the relation between carbon stocks and the amount of litterfall are usually mediated and carried out through changes in productivity. A study by Robert Amundson (2001) shows a trend of increasing soil carbon stocks in forests with an increase in average annual temperature, average annual precipitation and, accordingly, net primary productivity.
It is known that the productivity of woody plants in the northern hemisphere naturally decreases from south to north (Utkin, 1975). It is also shown that the amount of litterfall increases with decreasing latitude (Albrektson, 1988). Annual intake of litter in the North taiga forests varies within 0.9–2.5 t/ha (Nikonov, 1986), in the middle taiga — 2.1–3.9 t/ha (Kazimirov, 1977; Red’ko, 1984). More highly productive communities contribute to a greater intake of litterfall: according to literature data, the annual intake of litterfall in the forests of the coniferous-broadleaf subzone varied from 2.5 to 4.4 t/ha in the forests of the Moscow region (Karpachevsky, 1977), 3.1–4.4 t/ha in the forests of the Bryansk Polesie (Shabliy, 1990), and 3.9–12.2 t/ha in the forests of the North-Western Caucasus (Zon, 1950).
The litter quality of both coniferous and deciduous trees may vary depending on climatic conditions. The relation between the concentration of N and the average annual temperature and annual precipitation was revealed; it was found that for both coniferous and deciduous tree species, the total concentration of N in the litterfall increases with an increase in hydrothermal values (Berg, McClaugherty, 2020). It is believed that, at the regional level, temperature is the leading factor in determining the rate of litter decomposition (Meentemeyer, 1978; Hobbie, 1996). However, when studying the rate of decomposition of ground litter, taking into account the climatic gradient, it is noted that in boreal forests about 16% of decomposition can be explained by nitrogen concentration (Dyer et al., 1990). When studying the rate of decomposition of underground litterfall, taking into account climatic characteristics, it was shown that the chemical composition of the roots is the main regulator of decomposition processes, while climatic and environmental factors were of secondary importance (Silver, Miya, 2001). It is shown that the quality of litter can be a more significant predictor of soil organic horizon decomposition as compared to hydrothermal characteristics (Swift et al., 1979; Berg, 2000), especially at the initial stages of its decomposition (Canessa et al., 2021).
2.2. Local level
2.2.1. Interbiogeocenotic heterogeneity
Studies show that the diversity of tree species increases forest productivity due to greater spatial complementarity of tree crowns, which, in turn, provides a positive correlation between the diversity of tree species and the productivity of litterfall (Zheng et al., 2019). The amount of litterfall increased with increasing species richness. Data on the relation between age and amount of litterfall are contradictory. Some publications have noted a positive relation between age and amount of litterfall in the first 98 years of the development of the pine community with the subsequent flat trend (Chen et al., 2017); in other studies the amount of litterfall decreased with increasing age of the stand (Albrektson, 1988). It is also noted that the amount of litterfall may increase with increasing soil fertility (Albrektson, 1988). In coniferous-broadleaf forests the effect of age on the amount of litterfall was not seen (Huang et al., 2017).
Low-quality litter is characterized by a low content of bases, high acidity, high content of lignin and secondary metabolites, as well as a wide C/N ratio. The litter of coniferous trees has a low nitrogen content: for example, pine litter often contains less than 0.4% nitrogen (Berg, McClaugherty, 2020); the litter of boreal shrubs is rich in polyphenolic compounds (Wardle et al., 2003); and green mosses have a low content of nutrients (Hilli, 2013).
The high-quality litter is characterized by a high content of bases, low acidity, and narrow C/N ratio. Deciduous tree litter is rich in nitrogen: for example, birch litter contains 0.7%, beech — 0.9%, aspen — 1.0%, hornbeam — 1.1%, oak — 1.2%, maple — 1.3%, and lime — 1.5% (Simon et al., 2018). The richer is litter with nutrients, the faster is the soil organic horizon decomposed by soil biota, which leads to a decrease in its stock and, accordingly, carbon stocks in it.
Already in the 1990s it was recognized that the availability of nitrogen is the main determinant controlling the response of soil carbon to climate changes in ecosystems whose nitrogen is a limiting factor (Diaz et al., 1993; Nohrstedt, 1992). A number of studies have shown that the addition of nitrogen stimulates the decomposition of high-quality litter, but slows down or prevents the decomposition of low-quality litter (Knorr et al., 2005), which is due, on the one hand, to the suppression of the activity of lignolytic enzymes (Carreiro et al., 2000), and, on the other hand, to an increase in the number of microorganisms (Córdova et al., 2018). There is also evidence that nitrogen stabilizes organic matter in the soil (Neff et al., 2002; Swanston et al., 2004) and prevents the mineralization of carbon accumulated earlier (Hagedorn et al., 2003). Broadleaf species, in particular elm, oak and poplar, can be considered the land-reclamation species that accelerate the nutrient cycle in pine plantations (Polyakova, Billor, 2007).
When comparing decomposition rates of different plant groups, the influence of the functional characteristics of plants associated with phylogenetic groups was demonstrated. Faster decomposition of deciduous broadleaf species as compared to coniferous species, as well as a faster decomposition of grass species as compared to cereals, has been found. Slow decomposition rates of ferns and mosses have been noted (Cornwell et al., 2008).
However, the most common predictors of the decomposition rate of soil organic horizon are relative indicators like the C/N ratio, as well as the content of nutrients in the soil organic horizon (Zhang et al., 2008). Some European (Lovett et al., 2004; Reich et al., 2005; Oostra et al., 2006) and North American studies (Finzi et al., 1998; Neirynck et al., 2000; Dijkstra, Fitzhugh, 2003; Hagen-Thorn et al., 2004) of plants of the genera Fraxinus, Acer, Quercus and Fagus show the differences in carbon pools of the soil organic horizon and the C/N ratio as an indicator of accumulation rate of the soil organic horizon. Ash, maple and lime are combined into a group of plants with high-quality litter, that is, the litter with high nitrogen content, which leads to low accumulation of C in the soil organic horizon due to a high decomposition rate. Oak and beech have a relatively low content of C and N in the litterfall which results in low content of C and N in the forest soil organic horizon, high C/N ratio in the forest soil organic horizon, and low decomposition rate, which leads to an increase in pools of C and N in the forest soil organic horizon. When comparing coniferous species with deciduous ones, it was found that spruce has the highest C/N ratio and carbon stocks in the soil organic horizon (Vesterdal et al., 2008). At the same time, comparison of coniferous species with each other showed that the soil organic horizon of pine forests often has a much wider C/N ratio than that of spruce forests (Lukina et al., 2020). Several studies have confirmed the informative value of the lignin/N ratio of the soil organic horizon in predicting the rate of decomposition of the soil organic horizon between species (Gower, Son, 1992, Heim, Frey, 2004). The following lignin/N ratios were revealed (in descending order): spruce, beech > oak >> maple, lime >>> ash (Melillo et al., 1982; Lovett et al., 2004; Sariyildiz, Anderson, 2005; Kalbitz et al., 2006; Cotrufo et al., 2013).
High-quality litter, i. e. that enriched with nutrients and with a minimum C/N ratio and lignin content, usually decomposes faster than low-quality litter (with nutrient deficiency and a lot of lignin). However, the contribution of rapidly and slowly decomposing litter fractions to SOM accumulation is currently not fully clear (Castellano et al., 2015).
It is believed that slowly decomposing material of soil organic horizon contributes to the accumulation of carbon in the soil more than more rapidly decomposing material (Swift et al., 1979), especially in organogenic soil horizons, since low-quality litter is slowly processed by soil biota (Striganova, 1980; Prescott et al., 2000; Huang et al., 2020; etc.) and promotes the growth of fungi and their contribution to carbon stabilization on the mineral matrix (Six et al., 2004; Soares, Rousk, 2019). However, the results of other studies show that mixing slowly decomposing soil organic horizon with low-quality litter and rapidly decomposing soil organic horizon with high-quality litter contributes to higher efficiency of carbon transfer to soil mineral horizons (Cotrufo et al., 2013; Córdova et al., 2018), mainly due to an increase in DOC fluxes from the developed soil organic horizon (Fröberg et al., 2011) and by adding readily available nitrogen of rapidly decomposing litter fractions. It has been shown that the content of DOC, especially in the surface layers of the soil, is positively correlated with the decomposition rate of the soil organic horizon (Zhou et al., 2015). There are regular connections between litter stocks and the flux of DOC: in forests with a high proportion of deciduous trees with less developed soil organic horizon, less intensive removal of DOC was noted (Fröberg et al., 2011). There is also data on the relation between the qualitative and quantitative characteristics of DOC and root distribution.
Another important aspect of the impact of litter quality is the comparison of litter quality in the soil organic horizon of monodominant and mixed forests. It has been shown that forests with higher diversity, that is, high plant species density, have a narrower C/N ratio (Polyakova, Billor 2007; Huang et al., 2017). There are also studies on the positive effect of plant functional diversity on the decomposition of litter (Patoine et al., 2017), since the combined effect of litter of different quality can create special favorable conditions for the activity of soil biota. For example, a number of studies have shown that the poor quality of spruce and fir litterfall results in the accumulation of the soil organic horizon, which is a habitat for saprophages functionally related to it (Kuznetsova et al., 2019; Huang et al., 2020).
The joint influence of species is seen when comparing carbon stocks of different forest types (Framstad et al., 2013; Lukina et. al., 2020; Kuznetsova et al., 2021). The differences may be due to the different ratio of plants of the tree layer. In the soils of broadleaf forests, it was not possible to find significant differences between carbon accumulation in monocultures in common garden experiments (Vesterdal et al., 2008). The question of the combined effect of several species of woody plants on carbon stocks remains open. V. N. Shanin and colleagues (Shanin et al., 2014) studied the influence of the richness of boreal forests on their productivity and carbon dynamics and revealed that mixed forests are more productive than monodominant ones. With regard to coniferous-deciduous forests, it has been shown that a greater variety of tree species causes an increase in organic carbon stocks in the soil (Vesterdal et al., 2013). However, there are other estimates showing that the carbon stocks of the soil are more influenced by the identity of tree species as compared to their diversity (Dawud et al., 2016).
The higher the diversity of woody plant species, the more mixed the litter becomes. At the same time, the litter of conifers is decomposed faster in the presence of deciduous tree litter (Patoine et al., 2017), therefore, the quality of litter in general improves, which contributes to more intensive decomposition and an increase in carbon fluxes into mineral horizons. Manipulations with the ratio of species with different litter quality are common practice in climate-smart forestry (Mayer et al., 2020).
There are few studies on the relation between the diversity of vegetation under the canopy with ground cover and carbon dynamics. In boreal forests, there has been a significant increase in carbon stocks in forests where the contribution of grasses to the total projective cover exceeds 10% (Lukina et al., 2020). In coniferous-broadleaf forests, a positive relation between the content of soil carbon and the diversity of trees in the layer of sub-canopy vegetation was noted (Bakhshandeh-Navroud et al., 2018).
Unlike boreal forests, where mosses and dwarf shrubs mainly predominate in the ground cover and the addition of grasses significantly affects the processes of carbon accumulation, in coniferous-broadleaf forests, where grasses mainly predominate in the ground cover, their influence may be less pronounced (Kuznetsova et al., 2021).
- Intrabiogeocenotic heterogeneity
There are quite a lot of works evaluating carbon cycles in different mosaic elements (Orlova, Lukina, 2016; Lukina et al., 2018; Priputina et al., 2020; etc.). Humification of the leaf-based soil organic horizon in the gaps and below the crowns differed in winter and summer (Ni et al., 2015). Emission was 4 times higher in the gaps of old-aged spruce forests as compared to the space below the canopy (Karelin et al., 2017). The mass of needle litterfall was significantly higher (7.5%) directly under the tree crowns. Whereas the characteristics of the litter quality (acidity, content of N and other nutrients, C/N ratio) did not show any significant differences when comparing the spaces in below and between the crowns in 55-year-old pine forests of northwestern Germany, a number of studies show trends towards higher pH and nutrient content in spaces below the crowns in contrast to spaces between the crowns (Penne et al., 2010).
There are very few studies assessing the influence of different mosaic elements related to the distribution of underground parts of plants on both cycles and carbon pools (Liang et al., 2017; Sokol et. al., 2019). It is known that different types of trees have different patterns of root distribution in the soil profile. Root systems of the common spruce are of the surface type and are located mainly in the forest soil organic horizon (Puhe, 2003). In the mineral soil, low mass of roots was seen (<5 mm) at a depth of 0–20 cm under the spruce and beech as compared to the oak and ash (Oostra et al., 2006). It has also been reported that ash has relatively thinner roots at a depth of 16–30 cm than oak, whereas at a depth of 0–15 cm the situation is quite opposite (Ponti et al., 2004). Analysis of the qualitative composition of organic carbon taking into account the depth of the soil profile showed an increase in the content of lipids, the source of which was underground litterfall, as compared to the content of lipids, the source of which was ground litterfall (Nierop, 1998; Nierop et al., 2006; Feng, Simpson, 2007; Spielvogel et al., 2014; Angst et al., 2016). Using the example of both coniferous and deciduous forests, it was shown that root litterfall can make a comparable contribution to the soil carbon stock as compared to ground litterfall (Rasse et al., 2005).
Regulation of the migration of carbon compounds by atmospheric precipitation and soil waters within the boundaries of the soil profile may also be associated with the structural organization of biogeocenosis (Ershov, 2021). According to long-term observations, the intake of organic carbon compounds with atmospheric precipitation in northern taiga forests below pine crowns is 5-6 times higher than in spaces between the crowns throughout the vegetation season, which may also explain the differences in carbon concentrations in soil waters (Lukina et al., 2018; Ershov et al., 2019). Significant carbon removal with soil waters is typical of coniferous forests, especially in the spaces between the crowns (Fröberg et al., 2011; Lukina et al., 2018; Akkumuljacija…, 2018).
Thus, forest biodiversity may influence carbon cycles, on the one hand, through change in quality and quantity of litterfall, and, on the other hand, through change in physical environmental conditions like humidity or temperature. All this affects the change in activity of the soil biota. Vegetation composition determines quantity, quality and rate of decomposition of plant litter, its horizontal distribution and distribution of carbon compounds within the soil profile (Gleixner, 2013), which determines the formation of soil carbon pools.
- Existing estimates of carbon stocks in taiga and coniferous-broadleaf forests
3.1. Regional level
Russia accounts for more than 20% of the world’s forest cover and more than half of the world’s boreal forest resources. According to existing estimates, the soils of forest ecosystems account for about 46% of the total carbon stocks in the soil cover of Russia (Schepashchenko et al., 2013). At the same time, the contribution of forest lands in the Asian and European parts of the country is different and amounts to 48% and 37%, respectively, which reflects the level of forest cover of these territories.
Soil carbon stocks are closely related to the natural and climatic zone: the highest level of accumulation is characteristic of forests that formed under cool and humid conditions, whereas in warmer and drier climates, stocks decrease both on a global scale (Post et al., 1982; Jobbagy, Jackson, 2000) and on a (sub)regional scale (Burke et al., 1989; Alvarez, Lavado, 1998; Paul et al., 2002; Callesen et al., 2003; Baritz et al., 2010; Badgery et al., 2013; de Brogniez et al., 2014; Rossel et al., 2014; Hobley et al., 2015; Gray et al., 2016; Chestnyh et al., 2020).
Total carbon stocks in automorphic and semi-hydromorphic soils, taking into account the hydromorphic soils of swamps in the forest areas of the European-Ural part with an area of 181.13 × 106 ha, amount to 19.3 × 109 t C (Chestnyh et al., 2020). Of these, north taiga carbon stocks in the 0–30 cm layer, including the carbon stocks of the soil organic horizon, organogenic and mineral horizons, amount to 4.94 ± 2.01 × 109 t C, in the middle taiga forests — 2.92 ± 0.93 × 109 t C, and in southern taiga forests — 2.09 ± 1.80 × 109 t C. The lowest stocks were revealed in the zone of coniferous-broadleaf forests — 1.02 ± 0.67 × 109 t C. The highest average values were found in the soils of the northern taiga forests, if we take swamp forests into account. The minimum average values are typical for the coniferous-broadleaf area, which the authors explain with either climatic features and widely spread breaking new grounds, or lack of data on swamps in this area.
In automorphic soils, soil organic horizon on average accounts for 30% of the total stock of C in the 0–30 cm layer, whereas in layers of 0–50 cm and 0–100 cm, it decreases to 24% and 18%, respectively (Chernova et al., 2020). The average carbon stock of the soil organic horizon depends on the natural and climatic zone: there is a trend to decreasing stocks of the soil organic horizon and carbon stocks therein from the northern taiga subzone to the subzone of coniferous-broadleaf forests (Chestnyh et al., 2007; Kuznetsova et al., 2020). The average carbon stock of the soil organic horizon is 11 t/ha in the forests of the northern taiga, 10 t/ha in the forests of the middle taiga, and 7 t/ha in the more southern regions (Chestnyh et al, 2007). Total carbon stock in the forest floor throughout Russia ranges from 5.3 Pg С (Chestnyh et al., 2007) to 8.4 Pg С (Schepashchenko et al., 2013).
In contrast to carbon stocks of the soil organic horizon, more intensive carbon accumulation was revealed in the mineral horizons of automorphic soils of the southern regions as compared to the northern ones. For example, carbon stocks of the soil organic horizon in blueberry pine forests naturally decreased from 47±8 t/ha in the northern taiga to 8±1 t/ha in coniferous-broadleaf forests, and stocks in the 0–10 cm layer representative of the humus-accumulative horizon, on the contrary, increased from 8±1 to 18± 2 t/ha (Kuznetsova et al., 2020).
3.2. Local level
3.2.1. Interbiogeocenotic heterogeneity
In the boreal forests of the European part of Russia, the average carbon stock varies from 10.6 to 17.2 t/ha in organic horizons, while in the 30-centimeter layer (mineral layer) it varies from 46.6 to 122.2 t/ha (Rasporjazhenie…, 2018). The average carbon stock of the soil organic horizon ranges from 0.6 to 28 t/ha in pine forests, from 0.9 to 58 t/ha in spruce forests, from 1 to 29 t/ha in broadleaf forests, from 0.3 to 27.4 t/ha in birch forests, and from 0.7 to 19.9 t/ha in aspen and other soft-wooded broadleaf forests (Chestnyh et al., 2007). In the Komi Republic, in the north-east of the European part of Russia, the carbon stock in the one-meter layer of soil varied from 29 t/ha to 121 t/ha, depending on the type of soil (Dymov, 2018). In the Republic of Karelia, the stocks of soil carbon in the one-meter layer also varied significantly and amounted to 24–434 t/ha in pine forests and 39–402.4 t/ha in spruce forests depending on the type of soil and humidity (Bakhmet, 2018). The results of soil carbon assessments in the boreal forests of the Scandinavian countries indicate that the greatest diversity of soil carbon stocks associated with environmental factors is detected in organic horizons (Framstad et al., 2013). According to field data, the average national value of organic carbon stocks was 92 t/ha in forests with spruce predominance and 57 t/ha in forests with pine predominance (Stendahl, 2010). Modeling has demonstrated that the accumulation of organic carbon stocks is 22% higher in spruce forests than in pine forests under similar environmental conditions. In Norway, soil carbon stocks were higher in more productive forests than in less productive forests, which was believed to be due to different soil thickness (de Wit, 1999). In Finland, carbon stocks in the soil varied depending on the weight of the forest soil organic horizon, weather conditions and logging (State of…, 2012). It has been shown that a more productive forest accumulates more carbon in the soil of Finnish forests (Leskinen et al., 2020). In Canada, soil carbon stocks in boreal mixed forests on sandy loam (northeast Ontario) was on average 51 t/ha and amounted for up to 30% of the total carbon stocks, whereas the forest soil organic horizon accumulated 22 to 36 t C per ha. In the Canadian boreal biome, soils in forests of black spruce, that have a slow carbon cycle, stored more organic carbon than the soils of aspen forests. (Laganiere, 2013).
In the zone of coniferous-broadleaf forests of the European part of Russia, the average carbon stock ranges from 10.6 to 17.2 t/ha in organic horizons, while in the 30-centimeter soil layer it ranges from 46.6 to 122.2 t/ha (Rasporjazhenie…, 2018). In the secondary post-agrogenic lime-aspen mixed-herbs forests of the Moscow region, the carbon stock in the 0–60 cm layer was 88 t/ha (Baeva et al., 2017). In the forest biogeocenoses of the Middle Volga region in the soil layer of 0–50 cm, the carbon stock ranges from 12.8 to 439.5 t/ha depending on the type of forest, soil type and humidity (Demakov et al., 2018).
In the organic horizon of broadleaf forests of the Central Europe, low carbon stock was found under the beech (0.42 t/ha) and in lime forests (0.20 t/ha) (Langenbruch, 2012), whereas in hornbeam-oak forests it is up to 8.4 t/ha (Bruckman et al., 2016) and is about 6 t/ha in the organic horizon of spruce forests of the Western Black Sea region (Misir et al., 2012). In common garden experiments, carbon stocks of the soil organic horizon ranged from 1.8 t/ha in lime, maple and ash crops to 3.8 t/ha in oak and 4.5 t/ha in birch forests. Up to 14.5 t/ha of carbon accumulated under the spruce (Vesterdal et al., 2008).
Soil carbon stocks in the mineral layer of 0–20 cm amounted to 52 t/ha in beech forests and 45 t/ha in lime forests of Central Europe (Langenbruch, 2012), while in the 0–50 cm soil layer of hornbeam-oak forests it reached 77 t/ha (Bruckman et al., 2016), and in fir forests of the western Black Sea region — 155 t/ha (Misir et al., 2012). In common garden forests, carbon stocks in the 0–30 cm layer ranged from 61 t/ha in spruce and birch stands to 64–67 t/ha in maple and lime and 69–71 t/ha in oak and ash forests (Vesterdal et al., 2008).
Therefore, it was shown that forest soils of the temperate zone have a significant carbon stock of up to 100 Mg C/ha or more. However, the variability is very high for both organogenic and mineral soil horizons. The rate of soil carbon sequestration in these forests depends on the type of soil, previous carbon stock, species composition of vegetation and other natural and anthropogenic factors (Lal, Lorenz, 2012).
3.2.2. Intrabiogeocenotic heterogeneity
There are very few studies assessing carbon pools in different elements of the mosaic. When comparing spruce parcels, minimal carbon stocks were found in the oxalis and dead-cover parcels, where stocks on average amount to 13 t/ha in the upper mineral layer of 0–5 cm as compared to blueberry, green moss and sedge spruce parcels, where stocks amount to 17–18 t/ha (Podvezennaja, Ryzhova, 2010). An increased pool of the soil organic horizon in the near-trunk spaces was shown as compared to the spaces below and between the crowns (Podvezennaja, Ryzhova, 2010). The example of pine forests in northwestern Germany demonstrated a tendency to increasing carbon stocks of soil organic horizon from 35 ± 9 t/ha in the spaces below the crowns to 38 ± 9 t/ha in the spaces between the crowns (Penne et al., 2010).
CONCLUSION
Vegetation as the main source of organic matter entering the soil determines the possibility and rate of soil organic matter formation and accumulation. Three main ways of transferring carbon from the vegetation pool to the soil pool have been identified: processing of aboveground and underground litterfall by soil fauna and microbiota, association of organic matter with soil aggregates due to root litter and root exudates, leaching of dissolved organic carbon from living plants and forest soil organic horizon. At the same time, there are no estimates of the contribution of each of these mechanisms or their combined impact. It was shown that such mechanisms of soil organic matter fixation as physical protection of organic matter in soil aggregates and biochemical stability of plant compounds require attention.
Analysis of the current state makes it possible to identify three main mechanisms that determine the dynamics of soil carbon pools and are associated with vegetation: quantity and quality of litter of both individual species (species identity) and their joint influence (structural diversity of communities). Vegetation diversity affects carbon and nitrogen cycles through changes in biotic conditions (litter quality and quantity), on the one hand, and through changes in physical environmental conditions (humidity, temperature), on the other hand. The influence of all aspects of diversity is significant. There are a number of works showing the influence on carbon of tree species, age of the stand, structure of crowns and mosaic pattern of the biogeocenosis.
Due to a close relation between vegetation diversity and carbon stocks, it is possible to manage soil carbon pools with the introduction of climate-smart forestry. However, in order to properly manage the size of the soil carbon pool, deeper knowledge of the sequestering potential of soils and its main controlling factors is needed, where gaps in knowledge are known. For example, there are singular estimates of the contribution of the lower layers of vegetation to the variation of soil carbon stocks, rare are estimates of the effect of micromosaic pattern (structural diversity) of forests on the soil carbon pool, comparison of the influence of individual woody plants and their combined effect on the soil carbon pool. Estimates of the impact of vegetation regulation of volume and composition of precipitation penetrating through the vegetation canopy on the soil carbon pool are also rare, and there are next to none estimates of the contribution of combinations of various factors, including vegetation-related ones, to the regulation of soil carbon stocks.
Studies are mostly focused not on carbon pools, but on individual components of stock estimates, mainly the carbon content. There is an issue as well that is related to the limited number of estimates of soil carbon pools in the subzone of coniferous-broadleaf forests.
FINANCING
The study was performed within the framework of the state CEPF RAS assignment 121121600118-8and RFBR project No 20-34-90137.
REFERENCES
Akkumuljacija ugleroda v lesnyh pochvah i sukcessionnyj status lesov (Carbon accumulation in forest soils and the successive status of forests), Ed. by N. V. Lukina, Moscow: Tovarishhestvo nauchnyh izdanij KMK, 2018, 232 p.
Albrektson A., Needle litterfall in stands of Pinus sylvestris L. in Sweden, in relation to site quality, stand age and latitude, Scandinavian Journal of Forest Research, 1988, Vol. 3, No 1–4, pp. 333–342.
Alvarez R., Lavado R. S., Climate, organic matter and clay content relationships in the Pampa and Chaco soils, Argentina, Geoderma, 1998, Vol. 83, No 1–2, pp. 127–141.
Amelung W., Zhang X., Flach K. W., Zech W., Amino sugars in native grassland soils along a climosequence in North America, Soil Science Society of America Journal, 1999, Vol. 63, No 1, pp. 86–92.
Amundson R., The carbon budget in soils, Annual Review of Earth and Planetary Sciences, 2001, Vol. 29, No 1, pp. 535–562.
Angst G., John S., Mueller C. W., Kögel-Knabner I., Rethemeyer J., Tracing the sources and spatial distribution of organic carbon in subsoils using a multi-biomarker approach, Scientific reports, 2016, Vol. 6, No 1, pp. 1–12.
Angst G., Messinger J., Greiner M., Häusler W., Hertel D., Kirfel K., Mueller C. W. Soil organic carbon stocks in topsoil and subsoil controlled by parent material, carbon input in the rhizosphere, and microbial-derived compounds, Soil Biology and Biochemistry, 2018, Vol. 122, pp. 19–30.
Angst G., Mueller K. E., Kögel-Knabner I., Freeman K. H., Mueller C. W., Aggregation controls the stability of lignin and lipids in clay-sized particulate and mineral associated organic matter, Biogeochemistry, 2017, Vol. 132, No 3, pp. 307–324.
Angst G., Mueller K. E., Nierop K. G., Simpson M. J., Plant- or microbial-derived? A review on the molecular composition of stabilized soil organic matter, Soil Biology and Biochemistry, 2021, Vol. 156, No 1–3, pp. 108–189.
Angst Š., Mueller C. W., Cajthaml T., Angst G., Lhotáková Z., Bartuška M., … & Frouz J., Stabilization of soil organic matter by earthworms is connected with physical protection rather than with chemical changes of organic matter, Geoderma, 2017, Vol. 289, pp. 29–35.
Anohina N. A., Biogennye uglevodorody v pochvah parkovyh zon goroda Moskvy. Avtoref. diss. kand. biol. nauk (Biogenic hydrocarbons in the soils of park zones of the city of Moscow. Abstract of candidate’s thesis), Moscow: MSU, 2020, 25 p.
Badgery W. B., Simmons A. T., Murphy B. M., Rawson A., Andersson K. O., Lonergan V. E., van de Ven R., Relationship between environmental and land-use variables on soil carbon levels at the regional scale in central New South Wales, Australia, Soil Research, 2013, Vol. 51, No 8, pp. 645–656.
Baeva Y. I., Kurganova I. N., De Gerenyu V. L., Pochikalov A. V., Kudeyarov V. N., Changes in physical properties and carbon stocks of gray forest soils in the southern part of Moscow region during postagrogenic evolution, Eurasian soil science, 2017, Vol. 50, No 3, pp. 327–334.
Bakhmet O. N., Carbon deposits in soils of pine and spruce forests of Karelia, Contemporary Problems of Ecology, 2018, Vol. 11, No 7, pp. 697–703.
Bakhshandeh-Navroud B., Abrari Vajari K., Pilehvar B., Kooch Y., The interactions between tree-herb layer diversity and soil properties in the oriental beech (Fagus orientalis Lipsky) stands in Hyrcanian forest, Environ. Monit. Assess., 2018, Vol. 190, 425 p.
Bardgett R., The biology of soil: a community and ecosystem approach, Oxford university press, 2005.
Baritz R., Seufert G., Montanarella L., Van Ranst E., Carbon concentrations and stocks in forest soils of Europe, Forest Ecology and Management, 2010, Vol. 260, No 3, pp. 262–277.
Berg B., Berg M. P., Bottner P., Box E., Breymeyer A., De Anta R. C., … & de Santo A. V., Litter mass loss rates in pine forests of Europe and Eastern United States: some relationships with climate and litter quality, Biogeochemistry, 1993, Vol. 20, No 3, pp. 127–159.
Berg B., Litter decomposition and organic matter turnover in northern forest soils, Forest Еcology and Management, 2000, Vol. 133, No 1–2, pp. 13–22.
Berg B., McClaugherty C., Plant Litter. 4th ed. Cham, Switzerland: Springer, 2020. 332 p.
Blume H.-P., Fleige H., Horn R., Kandeler E., Kogel-Knabner I., Kretzschmar R., Stahr K., Wilke B.-M. Soil Science, first ed. Springer Berlin Heidelberg, Berlin Heidelberg, 2015, 630 р.
Brassard B. W. Chen H. Y., Bergeron Y., Paré D., Differences in fine root productivity between mixed‐ and single‐species stands, Functional Ecology, 2011, Vol. 25, No 1, pp. 238–246.
Brassard B. W., Chen H. Y., Cavard X., Laganière J., Reich P. B., Bergeron Y., Yuan Z., Tree species diversity increases fine root productivity through increased soil volume filling, Journal of Ecology, 2013, Vol. 101, No 1, pp. 210–219.
Bruckman D., Campbell D. R., Pollination of a native plant changes with distance and density of invasive plants in a simulated biological invasion, American journal of botany, 2016, Vol. 103, No 8, pp. 1458–1465.
Brussaard L., Biodiversity and ecosystem functioning in soil, Ambio, 1997, pp. 563–570.
Bull I. D. van Bergen P. F., Nott C. J., Poulton P. R., Evershed R. P., Organic geochemical studies of soils from the Rothamsted classical experiments — V. The fate of lipids in different long-term experiments, Organic geochemistry, 2000, Vol. 31, No 5, pp. 389–408.
Burke I. C., Yonker C. M., Parton W. J., Cole C. V., Flach K., Schimel D. S., Texture, climate, and cultivation effects on soil organic matter content in US grassland soils, Soil science society of America journal, 1989, Vol. 53, No 3, pp. 800–805.
Cadisch G., Giller K. E., Driven by natureplant litter quality and decomposition, Wallingford, Oxon, UK: CAB International, 1997, 409 p.
Callesen I., Liski J., Raulund‐Rasmussen K., Olsson M. T., Tau‐Strand L., Vesterdal L., Westman C. J., Soil carbon stores in Nordic well‐drained forest soils — Relationships with climate and texture class, Global change biology, 2003, Vol. 9, No 3, pp. 358–370.
Canessa R., van den Brink L., Saldaña A., Rios R. S., Hättenschwiler S., Mueller C. W., … & Bader M. Y., Relative effects of climate and litter traits on decomposition change with time, climate and trait variability, Journal of Ecology, 2021, Vol. 109, No 1, pp. 447–458.
Carreiro M. M. Sinsabaugh R. L., Repert D. A., Parkhurst D. F., Microbial enzyme shifts explain litter decay responses to simulated nitrogen deposition, Ecology, 2000, Vol. 81, No 9, pp. 2359–2365.
Carrington E. M., Hernes P. J., Dyda R. Y., Plante A. F., Six J., Biochemical changes across a carbon saturation gradient: lignin, cutin, and suberin decomposition and stabilization in fractionated carbon pools, Soil Biology and Biochemistry, 2012, Vol. 47, pp. 179–190.
Castellano M. J., Mueller K. E., Olk D. C., Sawyer J. E., Six J., Integrating plant litter quality, soil organic matter stabilization, and the carbon saturation concept, Global change biology, 2015, Vol. 21, No 9, pp. 3200–3209.
Chen H. Y. H., Brant A. N., Seedre M., Brassard B. W., Taylor A. R., The contribution of litterfall to net primary production during secondary succession in the boreal forest, Ecosystems, 2017, Vol. 20, No 4, pp. 830–844.
Chernova O. V., Ryzhova I. M., Podvezennaja M. A., Ocenka zapasov organicheskogo ugleroda lesnyh pochv v regional’nom masshtabe (Assessment of organic carbon stocks of forest soils on a regional scale), Pochvovedenie, 2020, No 3, pp. 340–350.
Chestnyh O. V., Grabovskij V. I., Zamolodchikov D. G., Uglerod pochv lesnyh rajonov Evropejsko-Ural’skoj chasti Rossii (Soil carbon in forest areas of the European-Ural part of Russia), Voprosy lesnoj nauki, 2020, Vol. 3, No 2, pp. 1–15.
Chestnyh O. V., Lyzhin V. A., Koksharova A. V., Zapasy ugleroda v podstilkah lesov Rossii (The Carbon Reserves in Litters of Forests in Russia), Lesovedenie, 2007, No 6, pp. 114–121.
Clemente J. S., Simpson M. J., Physical protection of lignin by organic matter and clay minerals from chemical oxidation, Organic geochemistry, 2013, Vol. 58, pp. 1–12.
Córdova S. C., Olk D. C., Dietzel R. N., Mueller K. E., Archontouilis S. V., Castellano M. J., Plant litter quality affects the accumulation rate, composition, and stability of mineral-associated soil organic matter, Soil Biology and Biochemistry, 2018, Vol. 125, pp. 115–124.
Cornwell W. K., Cornelissen J. H., Amatangelo K., Dorrepaal E., Eviner V. T., Godoy O., Quested H. M., Plant species traits are the predominant control on litter decomposition rates within biomes worldwide, Ecology letters, 2008, Vol. 11, No 10, pp. 1065–1071.
Cotrufo M. F., Wallenstein M. D., Boot C. M., Denef K., Paul E., The microbial efficiency‐matrix stabilization (MEMS) framework integrates plant litter decomposition with soil organic matter stabilization: do labile plant inputs form stable soil organic matter? Global change biology, 2013, Vol. 19, No 4, pp. 988–995.
Cusack D. F. Chou W. W., Yang W. H., Harmon M. E., Silver W. L., L. Team, Controls on long‐term root and leaf litter decomposition in neotropical forests, Global Change Biology, 2009, Vol. 15, No 5, pp. 1339–1355.
Dawud S. M., Raulund-Rasmussen K., Domisch T., Finér L., Jaroszewicz B., Vesterdal L., Is tree species diversity or species identity the more important driver of soil carbon stocks, C/N ratio, and pH? Ecosystems, 2016, Vol. 19, No 4, pp. 645–660.
De Brogniez D., Ballabio C., van Wesemael B., Jones R. J., Stevens A., Montanarella L., Topsoil organic carbon map of Europe [in:] Soil Carbon, Cham: Springer, 2014, pp. 393–405.
De Wit H. A., Kvindesland S. Carbon stocks in Norwegian forest soils and effects of forest management on carbon storage, Norsk institutt for skogforskning, 1999, 59 р.
Demakov Ju. P., Isaev A. V., Nureev N. B., Mitjakova I. I., Granicy i prichiny variabel’nosti zapasov gumusa v pochvah lesov Srednego Povolzh’ja (The boundaries and reasons for the variability of humus reserves in the soils of the forests of the Middle Volga region), Vestnik Povolzhskogo gosudarstvennogo tehnologicheskogo universiteta. Serija: Les. Jekologija. Prirodopol’zovanie, 2018, No 3, рр. 30–49.
Diaz S. Grime J. P., Harris J., McPherson E., Evidence of a feedback mechanism limiting plant response to elevated carbon dioxide, Nature, 1993, Vol. 364, No 6438, pp. 616–617.
Dijkstra F. A., Cheng W., Interactions between soil and tree roots accelerate long‐term soil carbon decomposition, Ecology Letters, 2007, Vol. 10, No 11, pp. 1046–1053.
Dijkstra F. A., Fitzhugh R. D., Aluminum solubility and mobility in relation to organic carbon in surface soils affected by six tree species of the northeastern United States, Geoderma, 2003, Vol. 114, No 1–2, pp. 33–47.
Dungait J. A. J., Hopkins D. W., Gregory A. S., Whitmore A. P., Soil organic matter turnover is governed by accessibility not recalcitrance, Global Change Biology, 2012, Vol. 18, No 6, pp. 1781–1796.
Dyer M. L., Meentemeyer V., Berg B., Apparent controls of mass loss rate of leaf litter on a regional scale: litter quality vs. climate, Scandinavian Journal of Forest Research, 1990, Vol. 5, No 1–4, pp. 311–323.
Dymov A. A., Pochvy poslerubochnyh, postpirogennyh i postagrogennyh lesnyh jekosistem severo-vostoka evropejskoj chasti Rossii, Avtoref. diss. doct. biol. nauk (Soils of post-cutting, post-pyrogenic and post-agrogenic forest ecosystems in the northeast of the European part of Russia. Abstract of doctor’s thesis), Moscow: MSU, 2018, 46 p.
Ershov V. V., Lukina N. V., Orlova M. A., Isaeva L. G., Smirnov V. Je., Gorbacheva T. T., Ocenka dinamiki sostava pochvennyh vod severotaezhnyh lesov pri snizhenii ajerotehnogennogo zagrjaznenija vybrosami medno-nikelevogo kombinata, Sibirskij jekologicheskij zhurnal, 2019, Vol. 26, No 1, pp. 119–132.
Ershov V. V., Monitoring sostava atmosfery i pochvennyh vod v lesnyh jekosistemah: osnovnye jetapy i perspektivy (Assessment of the dynamics of the composition of soil waters in northern taiga forests with a decrease in airborne industrial pollution by emissions from the copper-nickel plant), Voprosy lesnoj nauki, 2021, Vol. 4, No 1, pр 1–34.
Feng X., Simpson A. J., Simpson M. J., Chemical and mineralogical controls on humic acid sorption to clay mineral surfaces, Organic Geochemistry, 2005, Vol. 36, pp. 1553–1566.
Feng X., Simpson M. J., The distribution and degradation of biomarkers in Alberta grassland soil profiles, Organic Geochemistry, 2007, Vol. 38, No 9, pp. 1558–1570.
Finzi A. C., Van Breemen N., Canham C. D., Canopy tree–soil interactions within temperate forests: species effects on soil carbon and nitrogen, Ecological applications, 1998, Vol. 8, No 2, pp. 440–446.
Fox O. Vetter S., Ekschmitt K., Wolters V., Soil fauna modifies the recalcitrance-persistence relationship of soil carbon pools, Soil Biology and Biochemistry, 2006, Vol. 38, No 6, pp. 1353–1363.
Framstad E., de Wit H., Mäkipää R., Larjavaara M., Vesterdal L., Karltun E. Biodiversity, carbon storage and dynamics of old northern forest, Copenhagen: Nordic Council of Ministers, 2013, 130 p.
Fröberg M., Hansson K., Kleja D. B., Alavi Gh., Dissolved organic carbon and nitrogen leaching from Scots pine, Norway spruce and silver birch stands in southern Sweden, Forest ecology and management, 2011, Vol. 262, No 9, pp. 1742–1747.
Frouz J., Effects of soil macro- and mesofauna on litter decomposition and soil organic matter stabilization, Geoderma, 2018, Vol. 332, pp. 161–172.
Frouz J., Livečková M., Albrechtová J., Chroňáková A., Cajthaml T., Pižl V., Háněl L., Starý J., Baldrian P., Lhotáková Z., Is the effect of trees on soil properties mediated by soil fauna? A case study from post-mining sites, Forest Ecology and Management, 2013, Vol. 309, pp. 87–95.
Gentile R., Vanlauwe B., Six J., Litter quality impacts short‐ but not long‐term soil carbon dynamics in soil aggregate fractions, Ecological Applications, 2011, Vol. 21, No 3, pp. 695–703.
Geraskina A. P., Vlijanie dozhdevyh chervej raznyh morfo-jekologicheskih grupp na akkumuljaciju ugleroda v lesnyh pochvah (Influence of earthworms of different morpho-ecological groups on the accumulation of carbon in forest soils), Voprosy lesnoj nauki, 2020, Vol. 3, No 2, pp. 1–20.
Gielen B., Neirynck J., Luyssaert S., Janssens I. A., The importance of dissolved organic carbon fluxes for the carbon balance of a temperate Scots pine forest, Agricultural and Forest Meteorology, 2011, Vol. 151, No 3, pp. 270–278.
Gill R. A., Jackson R. B., Global patterns of root turnover for terrestrial ecosystems, The New Phytologist, 2000, Vol. 147, No 1, pp. 13–31.
Gleixner G., Soil organic matter dynamics: a biological perspective derived from the use of compound-specific isotopes studies, Ecological Research, 2013, Vol. 28, No 5, pp. 683–695.
Gmach M. R., Kaiser K., Cherubin M. R., Cerri C. E. P., Lisboa I. P., Vasconcelos A. L. S., Siqueira‐Neto M., Soil dissolved organic carbon responses to sugarcane straw removal, Soil Use and Management, 2020, Vol. 37, No 1, pp. 126–137.
Gower S. T., Son Y., Differences in soil and leaf litterfall nitrogen dynamics for five forest plantations, Soil Science Society of America Journal, 1992, Vol. 56, No 6, pp. 1959–1966.
Grandy A. S., Neff J. C., Molecular C dynamics downstream: the biochemical decomposition sequence and its impact on soil organic matter structure and function, Science of the Total Environment, 2008, Vol. 404, No 2–3, pp. 297–307.
Gray J. M., Bishop T. F. A., Smith P. L., Digital mapping of pre-European soil carbon stocks and decline since clearing over New South Wales, Australia, Soil Research, 2016, Vol. 54, No 1, pp. 49–63.
Gunina A., Kuzyakov Y., Sugars in soil and sweets for microorganisms: Review of origin, content, composition and fate, Soil Biology and Biochemistry, 2015, Vol. 90, pp. 87–100.
Gunina A., Ryzhova I., Dorodnikov M., Kuzyakov Y., Effect of plant communities on aggregate composition and organic matter stabilisation in young soils, Plant and Soil, 2015, Vol. 387, No 1, pp. 265–275.
Hagedorn F., Spinnler D., Siegwolf R., Increased N deposition retards mineralization of old soil organic matter, Soil Biology and Biochemistry, 2003, Vol. 35, No 12, pp. 1683–1692.
Hagen-Thorn A., Callesen I., Armolaitis K., Nihlgård B., The impact of six European tree species on the chemistry of mineral topsoil in forest plantations on former agricultural land, Forest ecology and management, 2004, Vol. 195, No 3, pp. 373–384.
Heim A., Frey B., Early stage litter decomposition rates for Swiss forests, Biogeochemistry, 2004, Vol. 70, No 3, pp. 299–313.
Hilli S., Significance of litter production of forest stands and ground vegetation in the formation of organic matter and storage of carbon in boreal coniferous forests [in:] Forest condition monitoring in Finland — National report (Eds. P. Merilä, S. Jortikka), The Finnish Forest Research Institute, 2013, URL: https://clck.ru/agP2L (November 21, 2021).
Hobbie S. E., Temperature and plant species control over litter decomposition in Alaskan tundra, Ecological monographs, 1996, Vol. 66, No 4, pp. 503–522.
Hobley E., Wilson B., Wilkie A., Gray J., Koen T., Drivers of soil organic carbon storage and vertical distribution in Eastern Australia, Plant and Soil, 2015, Vol. 390, No 1, pp. 111–127.
Huang W., Gonzalez G., Zou X., Earthworm abundance and functional group diversity regulate plant litter decay and soil organic carbon level: A global meta-analysis, Applied Soil Ecology, 2020, Vol. 150, pp. 1–15.
Huang Y., Ma Y., Zhao K., Niklaus P. A., Schmid B., He J. S., Positive effects of tree species diversity on litterfall quantity and quality along a secondary successional chronosequence in a subtropical forest, Journal of Plant Ecology, 2017, Vol. 10, No 1, pp. 28–35.
Ivanova E. A., Formirovanie i razlozhenie drevesnogo opada v lesnyh jekosistemah v fonovyh uslovijah i pri ajerotehnogennom zagrjaznenii (Formation and decomposition of tree litter in forest ecosystems under background conditions and during airborne industrial pollution), Voprosy lesnoj nauki, 2021, Vol. 4, No. 3, pp. 1–52.
Jandl G. Leinweber P., Schulten H. R., Ekschmitt K., Contribution of primary organic matter to the fatty acid pool in agricultural soils, Soil Biology and Biochemistry, 2005, Vol. 37, No 6, pp. 1033–1041.
Jandl R., Lindner M., Vesterdal L., Bauwens B., Baritz R., Hagedorn F., Byrne K. A., How strongly can forest management influence soil carbon sequestration? Geoderma, 2007, Vol. 137, No 3–4, pp. 253–268.
Jobbаgy E. G., Jackson R. B., The vertical distribution of soil organic carbon and its relation to climate and vegetation, Ecological applications, 2000, Vol. 10, No 2, pp. 423–436.
Joly F. X., Milcu A., Scherer‐Lorenzen M., Jean L. K., Bussotti F., Dawud S. M., … & Hättenschwiler S., Tree species diversity affects decomposition through modified micro‐environmental conditions across European forests, New Phytologist, 2017, Vol. 214, No 3, pp. 1281–1293.
Kaiser K., Guggenberger G., Haumaier L., Changes in dissolved lignin-derived phenols, neutral sugars, uronic acids, and amino sugars with depth in forested Haplic Arenosols and Rendzic Leptosols, Biogeochemistry, 2004, Vol. 70, No 1, pp. 135–151.
Kaiser K., Zech W., Dissolved organic matter sorption by mineral constituents of subsoil clay fractions, Journal of Plant Nutrition and Soil Science, 2000, Vol. 163, No 5, pp. 531–535.
Kalbitz K. Solinger S., Park J. H., Michalzik B., Matzner E., Controls on the dynamics of dissolved organic matter in soils: a review, Soil science, 2000, Vol. 165, No 4, pp. 277–304.
Kalbitz K., Kaiser K., Bargholz J., Dardenne P., Lignin degradation controls the production of dissolved organic matter in decomposing foliar litter, European Journal of Soil Science, 2006, Vol. 57, No 4, pp. 504–516.
Kalbitz K., Kaiser K., Contribution of dissolved organic matter to carbon storage in forest mineral soils, Journal of Plant Nutrition and Soil Science, 2008, Vol. 171, pp. 52–60.
Karavanova E. I., Dissolved organic matter: Fractional composition and sorbability by the soil solid phase (review of literature), Eurasian Soil Science, 2013, Vol. 46, No 8, pp. 833–844.
Karavanova E. I., Zolovkina D. F., Stepanov A. A., Interaction of the Water-Soluble Organic Substances of Coniferous Litter with Minerals and Horizons of Podzolic Soil and Podzols, Eurasian Soil Science, 2020, Vol. 53, No 9, pp. 1234–1246.
Karelin D. V., Pochikalov A. V., Zamolodchikov D. G., Jeffekt usilenija jemissii СO2 v oknah raspada lesov Valdaja (Effect of increased CO2 emission in the decay windows of Valdai forests), Izvestija Rossijskoj akademii nauk. Serija geograficheskaja, 2017, No 2, pp. 60–68.
Karpachevskij L. O., Pestrota pochvennogo pokrova v lesnom biogeocenoze (Diversity of soil cover in forest biogeocenosis), Moscow: Izd-vo mosk. un-ta, 1977, 312 p.
Kazimirov N. I., Obmen veshhestv i jenergii v sosnovyh lesah Evropejskogo Severa (Metabolism and energy in pine forests of the European North), Leningrad: Nauka, 1977, 301 p.
Kiem R., Kögel-Knabner I., Contribution of lignin and polysaccharides to the refractory carbon pool in C-depleted arable soils, Soil Biology and Biochemistry, 2003, Vol. 35, No 1, pp. 101–118.
Knorr M., Frey S. D., Curtis P. S., Nitrogen additions and litter decomposition: A meta‐analysis, Ecology, 2005, Vol. 86, No 12, pp. 3252–3257.
Kogut B. M., Semenov V. M., Ocenka nasyshhennosti pochvy organicheskim uglerodom (Assessment of soil organic carbon saturation), Bjulleten’ Pochvennogo instituta im. V. V. Dokuchaeva, 2020, No 102, pp. 103–124.
Kovalev I. V., Kovaleva N. O., Pul ligninovyh fenolov v pochvah lesnyh jekosistem (Pool of lignin phenols in soils of forest ecosystems), Lesovedenie, 2016, No 2, pp. 148–160.
Krishna M. P., Mohan M., Litter decomposition Pinе forest ecosystems: a review, Energy, Ecology and Environment, 2017, Vol. 2, No 4, pp. 236–249.
Kuznetsova A. I., Geraskina A. P., Lukina N. V., Smirnov V. E., Tikhonova E. V., Shevchenko N. E., Gornov A. V., Ruchinskaya E. V., Tebenkova D. N., Linking Vegetation, Soil Carbon Stocks, and Earthworms in Upland Coniferous–Broadleaf Forests, Forests, 2021, Vol. 12, Article 1179.
Kuznetsova A. I., Lukina N. V., Gornov A. V., Gornova M. V., Tikhonova E. V., Smirnov V. E., … Genikova N. V., Carbon Stock in Sandy Soils of Pine Forests in the West of Russia, Eurasian Soil Science, 2020, Vol. 53, pp. 1056–1065.
Kuznetsova A. I., Lukina N. V., Tikhonova E. V., Gornov A. V., Gornova M. V., Smirnov V. E., Geraskina A. P., Shevchenko N. E., Tebenkova D. N., Chumachenko S. I., Carbon Stock in Sandy and Loamy Soils of Coniferous–Broadleaved Forests at Different Succession Stages, Eurasian Soil Science, 2019, Vol. 52, No 7, pp. 756–768.
Kuzyakov Y., Domanski G., Carbon input by plants into the soil. Review, Journal of Plant Nutrition and Soil Science, 2000, Vol. 163, No 4, pp. 421–431.
Laganiere J., Paré D., Bergeron Y., Chen H. Y., Brassard B. W., Cavard X., Stability of soil carbon stocks varies with forest composition in the Canadian boreal biome, Ecosystems, 2013, Vol. 16, No 5, pp. 852–865.
Lal R., Lorenz K., Recarbonization of the Biosphere, Dordrecht: Springer, 2012, pp. 187–201.
Langenbruch C., Helfrich M., Flessa H., Effects of beech (Fagus sylvatica), ash (Fraxinus excelsior) and lime (Tilia spec.) on soil chemical properties in a mixed deciduous forest, Plant and Soil, 2012, Vol. 352, No 1, pp. 389–403.
Lauenroth W. K., Gill R., Turnover of root systems [in:] Root ecology, Berlin: Springer, 2003, рр. 61–89.
Lei P., Scherer-Lorenzen M., Bauhus J., The effect of tree species diversity on fine-root production in a young temperate forest, Oecologia, 2012, Vol. 169, No 4, pp. 1105–1115.
Leskinen P., Lindner M., Verkerk P. J., Nabuurs G. J., Van Brusselen J., Kulikova E., Hassegawa M., Lerink B., Russian forests and climate change. What Science Can Tell Us, 11, Finland, Joensuu: European Forest Institute, 2020, URL: https://doi.org/10.36333/wsctu11 (November 21, 2021).
Liang C., Schimel J. P., Jastrow J. D., The importance of anabolism in microbial control over soil carbon storage, Nature microbiology, 2017, Vol. 2, No 8, pp. 1–6.
Lovett G. M. Weathers K. C., Arthur M. A., Schultz J. C., Nitrogen cycling in a northern hardwood forest: do species matter? Biogeochemistry, 2004, Vol. 67, No 3, pp. 289–308.
Ludwig M. Achtenhagen J., Miltner A., Eckhardt K. U., Leinweber P., Emmerling C., Thiele-Bruhn S., Microbial contribution to SOM quantity and quality in density fractions of temperate arable soils, Soil Biology and Biochemistry, 2015, Vol. 81, pp. 311–322.
Lukina N. V., Ershov V. V., Gorbacheva T. T., Orlova M. A., Isaeva L. G., Tebenkova D. N., Assessment of soil water composition in the Northern taiga coniferous forests of background territories in the industrially developed region, Eurasian Soil Science, 2018, Vol. 51, No 3, pp. 277–289.
Lukina N. V., Tikhonova E. V., Orlova M. A., Bakhmet O. N., Kryshen A. M., Tebenkova D. N., Kuznetsova A. I., Smirnov V. E., Braslavskaya T. Y., Gornov A. V., Shashkov M. P., Isaeva L. G., Zukert N. V., Associations between forest vegetation and the fertility of soil organic horizons in northwestern Russia, Forest ecosystems, 2019, Vol. 6, No 1, Article 34.
Lukina N., Kuznetsova A., Tikhonova E., Smirnov V., Danilova M., Gornov A., Bakhmet O., Kryshen A., Tebenkova D., Shashkov M., Knyazeva S., Linking Forest Vegetation and Soil Carbon Stock in Northwestern Russia, Forests, 2020, Vol. 11, No 9, Article 979.
Lützow Mv., Kogel-Knabner I., Ekschmitt K., Matzner E., Guggenberger G., Marschner B., Flessa H., Stabilization of Organic Matter in Temperate Soils: Mechanisms and Their Relevance under Different Soil Conditions — a Review, Eurasian Journal of Soil Science, 2006, Vol. 57, No 4, pp. 426–445.
Marschner B., Brodowski S., Dreves A., Gleixner G., Gude A., Grootes P. M., … & Wiesenberg G. L., How relevant is recalcitrance for the stabilization of organic matter in soils? Journal of plant nutrition and soil science, 2008, Vol. 171, No 1, pp. 91–110.
Mayer M., Prescott C. E., Abaker W. E., Augusto L., Cécillon L., Ferreira G. W., Vesterdal L., Tamm Review: Influence of forest management activities on soil organic carbon stocks: A knowledge synthesis, Forest Ecology and Management, 2020, Vol. 466, pp. 118–127.
Meentemeyer V., Macroclimate and lignin control of litter decomposition rates, Ecology, 1978, Vol. 59, No 3, pp. 465–472.
Melillo J. M., Aber J. D., Muratore J. F., Nitrogen and lignin control of hardwood leaf litter decomposition dynamics, Ecology, 1982, Vol. 63, No 3, pp. 621–626.
Mikutta R., Turner S., Schippers A., Gentsch N., Meyer-Stüve S., Condron L. M., Guggenberger G., Microbial and abiotic controls on mineral-associated organic matter in soil profiles along an ecosystem gradient, Scientific reports, 2019, Vol. 9, No 1, pp. 1–9.
Misir M., Misir N., Erkut S., Estimations of total ecosystem biomass and carbon storage for fir (Abies nordmanniana S. subsp. bornmülleriana (Mattf.)) forests (Western Black Sea Region), Kastamonu University Journal of Forestry Faculty, 2012, Vol. 12, No 3, pp. 60–64.
Neff J. C. Townsend A. R., Gleixner G., Lehman S. J., Turnbull J., Bowman W. D., Variable effects of nitrogen additions on the stability and turnover of soil carbon, Nature, 2002, Vol. 419, No 6910, pp. 915–917.
Neirynck J., Mirtcheva S., Sioen G., Lust N., Impact of Tilia platyphyllos Scop., Fraxinus excelsior L., Acer pseudoplatanus L., Quercus robur L. and Fagus sylvatica L. on earthworm biomass and physico-chemical properties of a loamy topsoil, Forest Ecology and Management, 2000, Vol. 133, No. 3, pp. 275–286.
Ni X., Yang W., Tan B., He J., Xu L., Li H., Wu F., Accelerated foliar litter humification in forest gaps: dual feedbacks of carbon sequestration during winter and the growing season in an alpine forest, Geoderma, 2015, Vol. 241, pp. 136–144.
Nierop K. G. J., Origin of aliphatic compounds in a forest soil, Organic geochemistry, 1998, Vol. 29, No 4, P. 1009–1016.
Nierop K. G. J., Preston C. M., Verstraten J. M., Linking the B ring hydroxylation pattern of condensed tannins to C, N and P mineralization. A case study using four tannins, Soil Biology and Biochemistry, 2006, Vol. 38, No 9, pp. 2794–2802.
Nikonov V. V., Zapasy i sostav podstilok vtorichnyh sosnjakov na severnom predele proizrastanija (Stocks and composition of litter of secondary pine forests at the northern limit of growth), Pochvovedenie, 1986, No 6, pp. 79–88.
Nohrstedt H. Ö., Soil water chemistry as affected by liming and im fertilization at two Swedish coniferous forest sites, Scandinavian Journal of Forest Research, 1992, Vol. 7, No 1–4, pp. 143–153.
Oostra S., Majdi H., Olsson M., Impact of tree species on soil carbon stocks and soil acidity in southern Sweden, Scandinavian Journal of Forest Research, 2006, Vol. 21, No 5, pp. 364–371.
Orlova M. A. Lukina N. V., Smirnov V. Je., Artemkina N. A., Vlijanie eli na kislotnost’ i soderzhanie jelementov pitanija v pochvah severotaezhnyh el’nikov kustarnichkovo-zelenomoshnyh (Effect of spruce on acidity and nutrient content in the soils of northern taiga dwarf-green moss spruce forests), Pochvovedenie, 2016, No 11, pp. 1355–1367.
Patoine G., Thakur M. P., Friese J., Nock C., Hönig L., Haase J., Scherer-Lorenzen M., Eisenhauer N., Plant litter functional diversity effects on litter mass loss depend on the macro-detritivore community, Pedobiologia, 2017, Vol. 65, pp. 29–42.
Paul K. I., Polglase P. J., Nyakuengama J. G., Khanna P. K., Change in soil carbon following afforestation, Forest ecology and management, 2002, Vol. 168, No 1–3, pp. 241–257.
Penne C., Ahrends B., Deurer M., Böttcher J., The impact of the canopy structure on the spatial variability in forest floor carbon stocks, Geoderma, 2010, Vol. 158, No 3–4, pp. 282–297.
Pérez-Harguindeguy N., Díaz S., Cornelissen J. H., Vendramini F., Cabido M., Castellanos A., Chemistry and toughness predict leaf litter decomposition rates over a wide spectrum of functional types and taxa in central Argentina, Plant and soil, 2000, Vol. 218, No 1, pp. 21–30.
Podvezennaja M. A., Ryzhova I. M., Zavisimost’ variabel’nosti zapasov ugleroda v pochve ot prostranstvennoj struktury rastitel’nogo pokrova lesnyh biogeocenozov (Dependence of the variability of carbon stocks in soil on the spatial structure of the vegetation cover of forest biogeocenoses), Vestnik Moskovskogo universiteta, Serija 17, Pochvovedenie, 2010, No 4, pp. 3–9.
Polyakova O., Billor N., Impact of deciduous tree species on litterfall quality, decomposition rates and nutrient circulation in pine stands, Forest Ecology and Management, 2007, Vol. 253, No 1–3, pp. 11–18.
Ponti F., Minotta G., Cantoni L., Bagnaresi U., Fine root dynamics of pedunculate oak and narrow-leaved ash in a mixed-hardwood plantation in clay soils, Plant and Soil, 2004, Vol. 259, No 1, pp. 39–49.
Post W. M., Emanuel W. R., Zinke P. J., Stangenberger A. G., Soil carbon pools and world life zones, Nature, 1982, Vol. 298, No 5870, pp. 156–159.
Prescott C. E., Litter decomposition: what controls it and how can we alter it to sequester more carbon in forest soils? Biogeochemistry, 2010, Vol. 101, No 1, pp. 133–149.
Prescott C. E., Zabek L. M., Staley C. L., Kabzems R., Decomposition of broadleaf and needle litter in forests of British Columbia: influences of litter type, forest type, and litter mixtures, Canadian Journal of Forest Research, 2000, Vol. 30, pp. 1742–1750.
Priputina I. V., Frolova G. G., Shanin V. N., Mjakshina T. N., Grabarnik P. Ja., Raspredelenie organicheskogo veshhestva i azota v dernovo-podburah Prioksko-Terrasnogo zapovednika i ego svjaz’ so strukturoj lesnyh fitocenozov (Distribution of organic matter and nitrogen in the soddy-podburs of the Prioksko-Terrasny reserve and its relationship with the structure of forest phytocenoses), Pochvovedenie, 2020, No 8, pp. 921–933.
Puhe J., Growth and development of the root system of Norway spruce (Picea abies) in forest stands — a review, Forest ecology and management, 2003, Vol. 175, No 1–3, pp. 253–273.
Quenea K., Derenne S., Largeau C., Rumpel C., Mariotti A., Variation in lipid relative abundance and composition among different particle size fractions of a forest soil, Organic Geochemistry, 2004, Vol. 35, No 11–12, pp. 1355–1370.
Rasporjazhenie Ministerstva prirodnyh resursov i jekologii RF ot 30 ijunja 2017 g. № 20-r “O metodicheskih ukazanijah po kolichestvennomu opredeleniju ob#ema pogloshhenija parnikovyh gazov” (Order of the Ministry of Natural Resources and Environment of the Russian Federation of June 30, 2017 No 20-r “On methodological guidelines for the quantitative determination of the volume of absorption of greenhouse gases”), URL: https://www.garant.ru/ products/ipo/prime/doc/71612096/ ( July 07, 2021).
Rasse D. P., Rumpel C., Dignac M. F., Is soil carbon mostly root carbon? Mechanisms for a specific stabilization, Plant and soil, 2005, Vol. 269, No 1, pp. 341–356.
Red’ko G. I., Lindulovskaja listvennichnaja roshha: uchebnoe posobie (Lindulovskaya larch grove: study guide), Leningrad: LTA, 1984, 96 p.
Reich P. B., Oleksyn J., Modrzynski J., Mrozinski P., Hobbie S. E., Eissenstat D. M., … & Tjoelker M. G., Linking litter calcium, earthworms and soil properties: a common garden test with 14 tree species, Ecology letters, 2005, Vol. 8, No 8, pp. 811–818.
Rossel R. A., Webster R., Bui E. N., Baldock J. A., Baseline map of organic carbon in Australian soil to support national carbon accounting and monitoring under climate change, Global Change Biology, 2014, Vol. 20, No 9, pp. 2953–2970.
Sariyildiz T., Anderson J. M., Variation in the chemical composition of green leaves and leaf litters from three deciduous tree species growing on different soil types, Forest Ecology and Management, 2005, Vol. 210, No 1–3, pp. 303–319.
Schulp C. J. E., Nabuurs G. J., Verburg P. H., de Waal R. W., Effect of tree species on carbon stocks in forest floor and mineral soil and implications for soil carbon inventories, Forest ecology and management, 2008, Vol. 256, No 3, pp. 482–490.
Semenov V. M., Ivannikova L. A., Tulina A. S., Stabilizacija organicheskogo veshhestva v pochve (Stabilization of organic matter in soil), Agrohimija, 2009, No 10, pp. 77–96.
Semenov V. M., Kogut B. M., Stepanov A. L., Mamontov A. G., Pochvennoe organicheskoe veshhestvo (Soil organic matter), Мoscow: GЕОS, 2015, 233 p.
Shablij I. V., Formirovanie dubovo-sosnovyh nasazhdenij v uslovijah svezhih sudubrav Juzhnoj chasti Poles’ja i Severnoj lesostepi, Avtoref. diss. kand. biol. nauk (Formation of oak-pine plantations in fresh soil conditions in the southern part of Polesie and Northern forest-steppe, Abstract of candidate’s thesis) Kiev, 1990, 25 p.
Shanin V., Komarov A., Mäkipää R., Tree species composition affects productivity and carbon dynamics of different site types in boreal forests, European Journal of Forest Research, 2014, Vol. 133, pp. 273–286.
Schepashchenko D. G., Muhortova L. V., Shvidenko A. Z., Vedrova Je. F., Zapasy organicheskogo ugleroda v pochvah Rossii (Organic carbon stocks in soils of Russia), Pochvovedenie, 2013, No 2, pp. 123–123.
Silver W. L., Miya R. K., Global patterns in root decomposition: comparisons of climate and litter quality effects, Oecologia, 2001, Vol. 129, No 3, pp. 407–419.
Simon J., Dörken V. M., L.-M.-Arnold A., Adamczyk B., Environmental conditions and species identity drive metabolite levels in green leaves and leaf litter of 14 temperate woody species, Forests, 2018, Vol. 9, No 12, Article 775.
Six J. Bossuyt H., Degryze S., Denef K., A history of research on the link between (micro) aggregates, soil biota, and soil organic matter dynamics, Soil and tillage research, 2004, Vol. 79, No 1, pp. 7–31.
Six J., Conant R. T., Paul E. A., Paustian K., Stabilization mechanisms of soil organic matter: implications for C-saturation of soils, Plant and soil, 2002, Vol. 241, No 2, pp. 155–176.
Smolander A., Kitunen V., Soil microbial activities and characteristics of dissolved organic C and N in relation to tree species, Soil Biology and Biochemistry, 2002, Vol. 34, No 5, pp. 651–660.
Soares M., Rousk J., Microbial growth and carbon use efficiency in soil: links to fungal-bacterial dominance, SOC-quality and stoichiometry, Soil Biology and Biochemistry, 2019, Vol. 131, pp. 195–205.
Sokol N. W., Bradford M. A., Microbial formation of stable soil carbon is more efficient from belowground than aboveground input, Nature Geoscience, 2019, Vol. 12, No 1, pp. 46–53.
Spielvogel S., Prietzel J., Leide J., Riedel M., Zemke J., Kögel-Knabner I., Distribution of cutin and suberin biomarkers under forest trees with different root systems, Plant and soil, 2014, Vol. 381, No 1, pp. 95–110.
State of Finland’s Forests 2012. Criterion 1. Forest resources. Carbon stock on forest land (1.4). URL: http://www.metla.fi/metinfo/sustainability/c1-carbon-stock.htm (July 7, 2021)
Stendahl J., Johansson M. B., Eriksson E., Nilsson A., Langvall O., Soil organic carbon in Swedish spruce and pine forests — differences in stock levels and regional patterns, Silva Fennica, 2010, Vol. 44, No 1, pp. 5–21.
Striganova B. R., Pitanie pochvennyh saprofagov, (Nourishment of soil saprophages), Moscow: Nauka, 1980, Chapter 1, pp. 8–15.
Swanston C. Homann P. S., Caldwell B. A., Myrold D. D., Ganio L., Sollins P., Long-term effects of elevated nitrogen on forest soil organic matter stability, Biogeochemistry, 2004, Vol. 70, No 2, pp. 229–252.
Swift M. J., Heal O. W., Anderson J. M., Anderson J. M., Decomposition in terrestrial ecosystems, Vol. 5, Berkeley: Univ. of California Press, 1979, 372 р.
Thevenot M., Dignac M. F., Rumpel C., Fate of lignins in soils: a review, Soil Biology and Biochemistry, 2010, Vol. 42, No 8, pp. 1200–1211.
Totsche K. U., Amelung W., Gerzabek M. H., Guggenberger G., Klumpp E., Knief C., Lehndorff E., Mikutta R., Peth S., Prechtel A., Ray N., Kogel-Knabner I., Microaggregates in soils, Journal of Plant Nutrition and Soil Science, 2018, Vol. 181, No 1, pp. 1–33.
Utkin A. I. Biologicheskaja produktivnost’ lesov (metody izuchenija i rezul’taty), (Biological productivity of forests (research methods and results)), Lesovedenie i lesovodstvo, Vol. 1, 1975, pp. 9–190.
Vesterdal L., Clarke N., Sigurdsson B. D., Gundersen P., Do tree species influence soil carbon stocks in temperate and boreal forests? Forest Ecology and Management, 2013, Vol. 309, P. 4–18.
Vesterdal L., Schmidt I. K., Callesen I., Nilsson L. O., Gundersen P., Carbon and nitrogen in forest floor and mineral soil under six common European tree species, Forest. Ecol. Manag, 2008, Vol. 255, No 1, pp. 35–48.
Wardle D. A., Nilsson M. C., Zackrisson O., Gallet C., Determinants of litter mixing effects in a Swedish boreal forest, Soil Biology and Biochemistry, 2003, Vol. 35, pp. 827–835.
Wiesmeier M., Urbanski L., Hobley E., Lang B., von Luetzow M., Marin-Spiotta E., van Wesemael B., Rabot E., Ließ M., Garcia-Franco N., Wollschläger U., VogelfIngrid H.-J., Kögel-Knabner I., Soil organic carbon storage as a key function of soils — a review of drivers and indicators at various scales, Geoderma, 2019, Vol. 333, pp. 149–162.
Wolters V., Invertebrate control of soil organic matter stability, Biology and fertility of Soils, 2000, Vol. 31, No 1, pp. 1–19.
Xiao C., Bolton R., Pan W. L., Lignin from rice straw Kraft pulping: Effects on soil aggregation and chemical properties, Bioresource technology, 2007, Vol. 98, No 7, pp. 1482–1488.
Zhang D. Hui D., Luo Y., Zhou G., Rates of litter decomposition in terrestrial ecosystems: global patterns and controlling factors, Journal of Plant Ecology, 2008, Vol. 1, No 2, pp. 85–93.
Zheng L. T., Chen H. Y. H., Yan E. R., Tree species diversity promotes litterfall productivity through crown complementarity in subtropical forests, Journal of Ecology, 2019, Vol. 107, No 4, pp. 1852–1861.
Zhou W. J. Sha L. Q., Schaefer D. A., Zhang Y. P., Song Q. H., Tan Z. H., … & Guan H. L., Direct effects of litter decomposition on soil dissolved organic carbon and nitrogen in a tropical rainforest, Soil Biology and Biochemistry, 2015, Vol. 81, pp. 255–258.
Zonn S. V., Gorno-lesnye pochvy severo-zapadnogo Kavkaza (Mountain forest soils of the northwestern Caucasus), Leningrad: Izd-vo AN SSSR, 1950, pp. 55–145.
Reviewer: Candidate of Agricultural Sciences L. G. Isaeva